May 28th, 2024
Written by: Catrina Hacker
Neuroscientists still have a lot to learn about the brain. Before the end of the 20th century, measuring human brain activity was relatively difficult, so neuroscientists interested in untangling the brain’s mysteries measured brain activity in animals instead, with the hope that it could teach them something about how human brains work. However, in the last few decades, a new method has changed the game. Using this method, neuroscientists and doctors can get precise images of the human brain in living patients, and even estimates of brain activity, revolutionizing the way that neuroscientists study the brain. This method is called magnetic resonance imaging (MRI),and it has become widely available to neuroscientists, opening a path to answers to previously unanswerable questions.
A hundred years ago, it would have been hard to believe that one day we would have such easy access to high-resolution pictures of the brains of living people, but today a neuroscience researcher can image the brain in just a few short minutes. In this post, I’ll break down how MRI works, how it has already impacted neuroscience research, and what the future of using MRI to study the brain may look like.
How does MRI work?
Understanding how an MRI scanner gets a picture of the brain starts with some physics. An MRI scanner (Figure 1) creates a temporary magnetic field and then measures how your body’s molecules respond when the magnetic field is removed in order to piece together a brain scan1. This works because it measures signals from hydrogen atoms that are present in the water throughout your brain (the H of H2O). Hydrogen atoms normally face random directions, but the magnetic field causes many of them to align in the same direction. When the magnetic field is removed, the hydrogen atoms fall back to random directions, which emits a small signal that can be measured by the scanner. This signal can be used to reconstruct an image of the brain, like fitting pieces into a puzzle to uncover the bigger picture. The patient wears a head coil over their head (Figure 1) to help focus and capture these small signals to build a picture of the brain. The resulting images allow doctors and researchers to measure things like the size of different parts of the brain and to visualize brain damage and tumors.
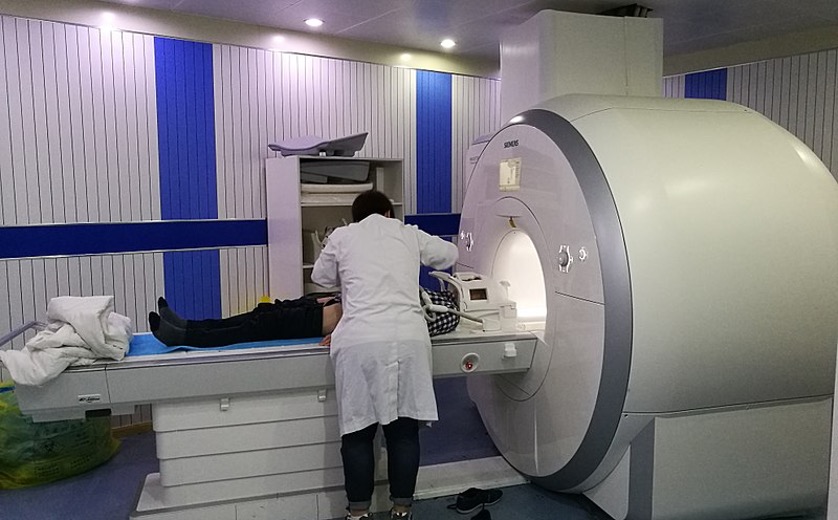
While putting the pieces of the puzzle together to get one big picture of the brain has been a helpful tool for neuroscientists, they need to know what each of those pieces can do to understand how the brain works. This means they need a way to measure brain activity. A powerful extension of MRI that allows researchers to do this is called functional MRI, abbreviated as fMRI. fMRI goes one step beyond an MRI, which just takes pictures of the brain, to indirectly measure neural activity.
fMRI works by measuring how a molecule that carries oxygen, called hemoglobin, is impacted by the magnetic field the MRI scanner creates2. Hemoglobin is your body’s oxygen delivery system, riding along the highway system of your blood vessels. When a particular brain area is being used, it recruits more oxygen, carried on the backs of hemoglobin molecules, to help that brain area do what it needs to do. You can think about it like how you might suck in an extra big breath of air when working out. Hemoglobin that is carrying oxygen responds differently to the magnetic field created by the MRI scanner than hemoglobin that has already “dropped off” its oxygen to the brain region that needs it. fMRI takes advantage of this difference to identify parts of the brain where oxygen is being delivered as a proxy for how hard the neurons in that brain region are working. This is called the Blood Oxygen Level Dependent (BOLD) signal. Because it’s measuring the delivery of oxygen and not the activity of neurons themselves, the BOLD signal is only an indirect measure of brain activity. However, this “best guess” at where neurons are being put to work has already helped neuroscientists learn a lot about what each of the pieces of the brain’s puzzle are doing.
What have we learned from fMRI research already?
One of the biggest impacts of fMRI has been the ability to look at neural activity across the entire brain at once. Most methods available to neuroscientists before fMRI only measured the activity of small groups of neurons in one or two places in the brain at once. The few methods that could look at the whole brain couldn’t tell precisely where neural activity was coming from. Now, using fMRI, neuroscientists can study how different regions across the entire brain interact with each other. For example, neuroscientists have discovered a network of brain regions that are active when we’re doing nothing, which they call the default mode network3. This has led to more research about how differences in the default mode network may be able to explain things like ADHD4 or depression5.
The widespread use of fMRI to study human brain activity has also allowed neuroscientists to study things unique to humans that are harder to study in animal models. For example, a recent paper used fMRI to study the brains of dozens of people who speak 45 different languages. The research group found a network of brain regions that support language processing that was present no matter what language someone speaks6. This would be impossible to study in animals who don’t have language like humans. Another example is in studying how music is processed by the brain. One recent study used fMRI to look at brain activity in people who learned to play music and those who hadn’t. They found that regardless of experience making music, all people have a part of their brain that responds specifically to music and not to other kinds of sounds7. Once again, this is a question that could not be directly answered without the use of fMRI, because while animals can listen to music, they can’t learn to play it.
What could fMRI help neuroscientists uncover moving forward?
As you’ve seen, fMRI has already shaped our understanding of the human brain in many ways. Even so, there are a lot of exciting new directions for fMRI research that are allowing researchers to answer even more interesting questions. For example, the fact that fMRI is easily repeatable allows neuroscientists to look beyond neural activity during specific tasks toward longer processes like brain development. As we grow and develop, it’s not just the size of our brains that change, and fMRI has been an incredible tool for charting and exploring these changes. Recent fMRI research reveals that the activity of our brains at rest as well as how different regions of the brain interact with each other change across the lifespan8,9. Moreover, by combining fMRI across the lifespan with measures of lifestyle and environment (e.g., a person’s involvement in sports and activities, substance use, screen time, neighborhood safety, etc.), scientists can get a better picture of the ways in which our environment impacts brain development and function.
Beyond studying how the healthy human brain develops, fMRI also has the potential to inform how we diagnose and treat brain dysfunction. For example, an ongoing effort at the Stanford Center for Precision Mental Health and Wellness is using fMRI to determine which brain networks (like the default mode network) are disrupted in patients with depression and anxiety10. The hope is that knowing exactly which pattern of brain network dysfunction a patient has could help match them to the best possible treatment. One challenge in studying mental health disorders has been that many people who have the same symptoms may have different underlying causes of their symptoms. fMRI could be a powerful solution to this problem to match patients to the treatments that will work best for them.
What’s the take home?
While there’s certainly a lot to be excited about when it comes to fMRI, it’s not a magic bullet for every question neuroscientists have. The BOLD signal is only an indirect measure of brain activity, so supplementing what we learn from fMRI with direct recordings of brain activity, typically done in animals, is an important part of closing the loop on our understanding of how the brain works. fMRI also struggles to measure smaller signals, meaning there are some things we may learn from direct recordings of neural activity that we can’t see using fMRI. Nevertheless, fMRI is a revolutionary tool in a neuroscientist’s toolbox that is helping neuroscientists get one step closer to solving the puzzle of how our brains function.
References
1. Plewes, D. B. & Kucharczyk, W. Physics of MRI: A primer. J. Magn. Reson. Imaging 35, 1038–1054 (2012).
2. Glover, G. H. Overview of Functional Magnetic Resonance Imaging. Neurosurg. Clin. N. Am. 22, 133–139 (2011).
3. Raichle, M. E. The Brain’s Default Mode Network. Annu. Rev. Neurosci. 38, 433–447 (2015).
4. Uddin, L. Q. et al. Network homogeneity reveals decreased integrity of default-mode network in ADHD. J. Neurosci. Methods 169, 249–254 (2008).
5. Zhou, H.-X. et al. Rumination and the default mode network: Meta-analysis of brain imaging studies and implications for depression. NeuroImage 206, 116287 (2020).
6. Malik-Moraleda, S. et al. An investigation across 45 languages and 12 language families reveals a universal language network. Nat. Neurosci. 25, 1014–1019 (2022).
7. Boebinger, D., Norman-Haignere, S. V., McDermott, J. H. & Kanwisher, N. Music-selective neural populations arise without musical training. J. Neurophysiol. 125, 2237–2263 (2021).
8. Sydnor, V. J. et al. Intrinsic activity development unfolds along a sensorimotor–association cortical axis in youth. Nat. Neurosci. 26, 638–649 (2023).
9. Luo, A. C. et al. Functional connectivity development along the sensorimotor-association axis enhances the cortical hierarchy. Nat. Commun. 15, 3511 (2024).
10. Williams, L. M. Systems and methods for detecting complex networks in MRI image data. (2020).
Cover Image by Dmitriy Gutarev from Pixabay
Figure 1 by Ptrump16 on Wikimedia Commons
Leave a comment